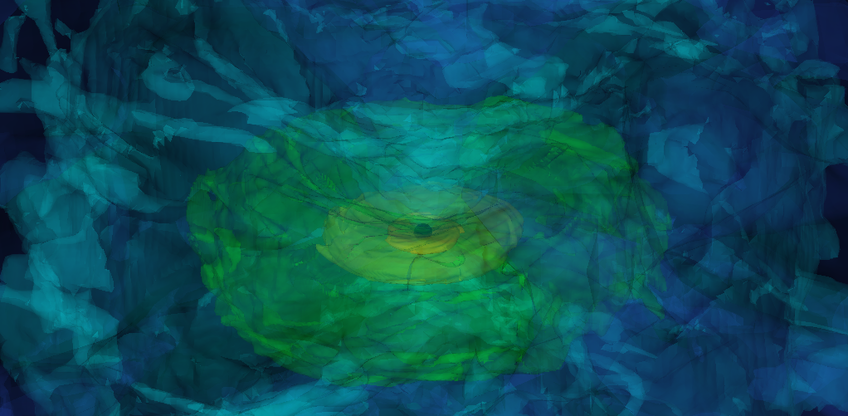
Computational Relativistic Astrophysics
Research in this department covers mergers of binary neutron stars and mixed binaries – a black hole and a neutron star – as well as stellar core collapse that form black holes. The department also focuses on studying more fundamental aspects of General Relativity using numerical tools.
Numerical relativity
To understand the formation process of black holes and neutron stars, and what happens when black holes and neutron stars merge, we have to fully solve Einstein's equation as well as the equations of motion for matter and electromagnetic fields, and radiation transfer equations in general relativity. It is generally not feasible to solve these nonlinear, partial differential equations analytically so we instead use numerical simulations on high-performance computers, for which we have been developing new methods in numerical relativity, since the 1990s. It is also feasible to accurately solve hydrodynamics/magnetohydrodynamics equations and to approximately solve radiation hydrodynamics equations for neutrino transport, using computational numerical methods.
For the numerical-relativistic calculations we use our supercomputer Sakura in Garching. With Sakura we have 11,600 CPU cores with a peak performance of 0,92 petaFLOP/s at our disposal.
We are now exploring a variety of dynamic and general relativistic phenomena such as:
- mergers of binary neutron stars and black hole-neutron star binaries
- stellar core collapse to a black hole and a surrounding torus
- formation of a supermassive black hole through the collapse of a supermassive star
- evolution of black holes and neutron stars by accretion of matter
- outflow and jets from black holes and neutron stars
These numerical relativity simulations play an important role in predicting gravitational waveforms, that are used for gravitational wave detection, and in exploring high-energy phenomena such as gamma-ray bursts an kilonovae. Numerical relativity is also robust for exploring nucleosynthesis of heavy elements and the nonlinear nature of gravity theories.
Predicting gravitational waveforms
Gravitational waves are emitted by general-relativistic and dynamical phenomena, if the system is not spherically symmetric. When powerful gravitational waves are emitted, they can be detected by gravitational-wave detectors such as advanced LIGO, advanced Virgo, and – in the future – also KAGRA, and Space gravitational wave detector, LISA. The advanced LIGO and Virgo detectors have already detected gravitational waves from binary black holes and binary neutron stars.
We expect that a large number of gravitational-wave sources will be observed by the gravitational-wave detectors in the near future. Upon detection, we have to identify the gravitational wave source and extract information such as mass, spin and distance from us from the data. To do this we have to prepare templates of gravitational waves emitted from the expected sources. We are now performing a large number of numerical-relativity simulations for the merger of binary neutron stars, black hole-neutron star binaries, stellar core collapse to a stellar-size and supermassive black hole, and so on, aiming at accurately predicting the corresponding gravitational waveforms.
Merger of binary neutron stars
Binary neutron star mergers are one of the most promising sources for gravitational-wave detectors like advanced LIGO, advanced Virgo, KAGRA and of short-hard gamma-ray bursts. To predict gravitational waveforms emitted by binary neutron star mergers and to theoretically explore the merger hypothesis of short-hard gamma-ray bursts, numerical relativity is the appropriate approach. In addition, the merger of binary neutron stars and associated mass ejection process is proposed as the primary environment for r-process nucleosynthesis. To explore this hypothesis, numerical relativity is again the appropriate theoretical approach. We have been working on this problem for the last two decades and discovered much about the merger process and resulting gravitational-waveforms.
Merger of black hole-neutron star binaries
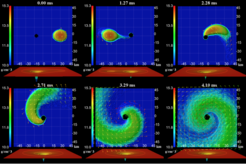
The merger of black hole-neutron star binaries is also among the most promising sources of gravitational waves and a promising candidate for the central engine of short-hard gamma-ray bursts. For this system, broadly speaking, there are two possible fates. In one scenario the neutron star is simply swallowed by the companion black hole, in the other the neutron star is tidally disrupted during the merger process. In the latter case, a wide variety of high-energy phenomena including gamma-ray bursts and mass ejection leading to transient electromagnetic emission are expected. We are exploring these phenomena using numerical relativity.
Merger of a low-mass black hole and neutron star leading to a black hole surrounded by an accretion disk
Gravitational collapse to a black hole
Massive stars evolve through a series of nuclear burning and eventually, in their centre, an iron core is formed. If the mass of the iron core (and surrounding layers) is sufficiently large, a black hole will be formed after the stellar core collapse. Massive stellar-size black holes discovered in binaries observed by advanced LIGO and advanced Virgo are likely to have been formed from such a process. However, the formation process of these black holes is not, yet, well understood.
Recent observations have shown that large galaxies usually have a supermassive black hole (SMBH) of a million to 10 billion solar masses in their centre. However, the formation process of an SMBH is still unknown: clarifying the formation process of an SMBH has been a long-standing problem in astrophysics.
We are investigating these problems in the framework of numerical relativity. Specifically, we perform a variety of numerical relativity simulations with different plausible progenitor stars aiming at clarifying the black-hole formation process. We then explore the possible signals from black hole formation such as gravitational waves, neutrinos, and electromagnetic waves, which may be detected in future observations leading to clarifying the formation process of black holes.
Magnetohydrodynamics simulation in General Relativity
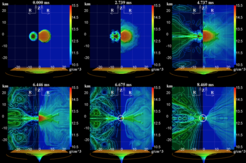
Magnetic fields play a crucial role in the processes of a wide variety of phenomena. It is quite likely that magnetic-field instabilities play an important role in the merger of binary neutron stars and in the evolution of accretion disks surrounding compact objects. For example, during the collision of two neutron stars, a shear layer is inevitably formed at the contact surface of the neutron stars. At such a shear surface, it is known that the Kelvin-Helmholtz instability occurs and a number of small vortices are generated. The vortex motion subsequently amplifies the magnetic-field strength through winding of the magnetic fields. After significant amplification of the magnetic field, turbulence is likely to occur, and the resulting turbulent viscosity could determine the evolution of the merger remnant because it contributes to angular momentum transfer and viscous heating. To understand these processes, we perform a magnetohydrodynamics simulation in general relativity. For accurate study, we need to correctly take into account the Kelvin-Helmholtz instability in the simulation. However, this is not a computationally easy task because the fast-growing mode of Kelvin-Helmholtz instability has very small scales and we have to resolve such a small-scale mode in numerical relativity. To date, no simulation has resolved this, and thus, no physical simulation has been done. In the future, we’ll aim to perform an extremely high-resolution simulation to fully clarify the merger process of binary neutron stars. Magnetic-field instabilities could also play a crucial role in the evolution of the merger remnant and accretion disks surrounding compact stars. There are many issues that should be attacked using magnetohydrodynamics simulations in general relativity.
Viscous hydrodynamics
One phenomenological way to take into account angular momentum transport and turbulent viscosity is to employ viscous hydrodynamics, with which we explore the merger remnant of binary neutron stars and the accretion disks surrounding the central compact objects.
Binary neutron stars in scalar-tensor theory
To date, we don’t have any evidence that general relativity is violated. However, the tests of general relativity have been performed in relatively weak gravitational environments such as in the solar system and with binary neutron stars of large orbital separation. Now that gravitational waves from a neutron star merger have been detected, we have the great opportunity of testing general relativity in a strong gravitational environment. For this test, it is important to predict what happens to the strong self-gravitating systems in an alternative theory of gravity. For one study, we performed simulations for the merger of binary neutron stars in a scalar-tensor theory of gravity.